The Step Recovery Diode (SRD) stands out as an intricate semiconductor device, characterized by its atypical doping patterns and its functional versatility in electronic applications. At the core of its structure lies an unusual doping concentration, where the doping density is astonishingly minimal near the junction area. This results in a notably low charge carrier density around the junction. One might deduce from this configuration that the charge storage in this area is negligible. This inherent property empowers the SRD with an impressive capability: swift transitioning from its ON state to the OFF state.
Traditional diodes are known to conduct when forward biased and to act as an open circuit in a reverse biased mode. The SRD behaves similarly at low frequencies. However, its distinctive feature emerges when transitioning from a forward bias to a reverse bias; it undergoes this change almost instantaneously. Despite this, at higher frequencies, this switching isn’t immediate. A minuscule delay occurs due to the residual charge carriers near the junction. Their density, albeit low, still demands a brief interval to be drained away from the junction as the frequency rises. This results in a phenomenon where the forward current persists for a short duration into the reverse cycle of the input voltage. This behavior is termed as “reverse snap-off,” giving the SRD its alternative name, the Snap-off Diode.
Despite being a less common member of the diode family, the SRD’s unique characteristics make it invaluable in specific scenarios. It’s sometimes referred to by other names such as charge-storage diode, memory varactor, and as previously mentioned, snap-off diode. Its ability to generate sharp, crisp pulses sets it apart. In the realm of microwave and radio frequency electronics, the SRD carves its niche. Its applications span across pulse generation, ultra-fast waveform creation, comb generation, and even high order frequency multiplication. Moreover, its capacity to operate at moderate power levels grants it an edge over several other RF technologies.
Step Recovery Diode is a remarkable semiconductor device, optimized for rapid switching and sharp pulse generation. Its unique structure and properties make it a crucial component in specific high-frequency applications, reinforcing its value in the ever-evolving landscape of electronic devices.
Step recovery diode construction
The Step Recovery Diode (SRD), at its core, bears a resemblance in its construction to the ordinary diode. What sets it apart, however, is the unique pattern of doping. Doping, in semiconductor parlance, refers to the intentional introduction of impurities into an intrinsic semiconductor to modify its electrical properties. In the SRD, this doping is characterized by a diminishing density as one approaches the junction of the diode. This deliberate gradient in doping intensity has a direct impact on the density of mobile charge carriers at the junction, causing it to decrease.
Drawing inspiration from the foundational Shockley diode, the SRD’s architecture consists of two interconnected pn-junctions. Additionally, it has a third terminal tailored to accept the input voltage. It’s this sophisticated design that bestows upon the SRD its unique dynamic switching characteristics, distinguishing it from the more traditional single PN junction diode. One might wonder about the benefits of such a design. The answer lies in the speed. With fewer charge carriers present within the junction’s region, the SRD boasts a swifter switching time, typically clocking in at just a few nanoseconds. This briskness is primarily due to the lesser volume of charge that needs to be dispatched swiftly as the diode transitions from a forward bias to a reverse bias.
In the realm of low frequencies, the SRD essentially mirrors the behavior of a standard diode, offering extremely low impedance – often as low as approximately 1 ohm. However, as it continues to operate, an intriguing phenomenon begins to manifest. The impedance starts to incrementally increase, attributable to the accumulation of charge at the junction. This accumulation introduces a slight delay during the initiation of a negative input cycle. Charge carriers, being present in the junction, require a finite time to be entirely drained off. This results in a perceptible, albeit minimal, current at the onset of the negative input phase.
Step Recovery Diode is a masterclass in semiconductor engineering, marrying the foundational principles of diode construction with the finesse of nuanced doping gradients. Its inherent design facilitates rapid switching, making it a prime candidate for applications demanding swift transitions and precision.
PIN SRD doping density
Doping density for the PIN SRD structure is decreasing as we move inside the structure, where at the P region end, doping intensity is lowering. At the I region, there is minor movement and no doping, which is almost 0. At the N region, we are observing doping intensity which decreases as we move towards the edge of the structure. Because of this behavior, charges which are moving on and off have a high velocity, so transition time decreases. While the diode is connected in forward bias, charges in the I region gradually decrease as they move to the N region. The PIN diode has more amount of charges due to having more space in the I layer than in the PN junction. The I region allows electrons and holes inside its structure because it doesn’t have any polarity. Forward bias generates gradual conduction. Reverse bias, at first instance, results in the snapping off of charge carriers, leading to a higher number of negative poles; this causes discontinuity. Charges recover in steps. That’s why the diode is called STEP RECOVERY diode.
Step recovery diode working principle
The Step Recovery Diode (SRD) is a fascinating semiconductor device known for its unique operational dynamics. At low frequencies, it manifests characteristics similar to a typical diode. Initially, when it’s operational, it showcases an extremely low impedance of about 1 ohm. However, as it continues functioning, a curious phenomenon takes place: the impedance starts to ascend due to the accumulation of charge at the diode’s junction.
But what happens when the diode encounters the negative cycle of input? This is where the SRD truly stands out. The charge carriers present at the junction don’t instantaneously drain off or “snap-off”. Instead, they require a brief moment, leading to a small surge of current at the onset of the negative input cycle. This distinct trait enables the SRD to have a minimal switching time, typically just a few nanoseconds. This brisk switching capability confers upon it the potential to generate sharp, well-defined pulses. Such pulses find critical applications in waveshaping circuits, emphasizing the importance of SRDs in electronics.
SRDs are frequently used in scenarios demanding repetitively pulsed operations, thanks to their swift recovery time. Their design allows them to endure high peak currents and voltages, earning them alternate names like peak current diodes or avalanche diodes. One of the pivotal features of the SRD is its sizable junction capacitance. This capacitance, formed by the depletion region between the two p-n junctions, has to be charged adequately before the diode can conduct. When the diode is subjected to reverse bias, this junction capacitance progressively charges until it hits a threshold, leading to what’s termed as the “avalanche breakdown.” During this phase, electrons traverse the diode uninhibited, inducing a substantial current flow and consequent heat generation.
An intriguing aspect of the SRD’s behavior is the sudden drop of reverse current to zero once the stored charge dissipates. This eliminates the tailing of the reverse current. Given its inherent ability to store a considerable volume of minority charge carriers during forward voltage application, it has garnered the moniker “charge storage diode.”
One of the standout characteristics of the SRD is its negative resistance. This might sound counterintuitive, but in the realm of electronics, it denotes that the diode conducts an increased current as its voltage diminishes. To truly grasp the SRD’s operational prowess, one needs to delve into the concept of negative resistance. It’s a scenario wherein a device, upon conducting current, sees a voltage drop across it, contrary to the standard behavior of voltage increment. This unique trait allows the SRD to potentially produce a negative voltage, which can be harnessed for energy. Consequently, in specific circuit configurations, the SRD can perform roles usually reserved for amplifiers or oscillators.
Drift step recovery diodes
The drift step recovery diode (DSRD) is a specialized semiconductor device, designed specifically for generating rapid high-voltage pulses on the scale of nanoseconds. With the capability of producing these pulses at a staggering repetition frequency reaching into the hundreds of kilohertz continuously and even surging to around 10 MHz in burst mode, the applications of DSRDs are broad and impactful. For instance, their utility in powering lasers, enabling Pockels cell switching, or assisting in ground-penetrating radar systems underscores their significance in advanced technological applications.
One defining feature of a DSRD is its operational mechanism. It operates by initially charging its pn junction when pumped with a low forward current, followed by a subsequent high reverse current pulse. This unique procedure triggers a rapid current cessation when the pn junction discharges, and by leveraging an inductor, this abrupt current halt can be transmuted into a swift voltage rise across the associated load.
At the core of the DSRD is its four-layered p+pnn+ structure, where the intricacies of the current-breaking process play out. While the junction remains central to its functionality, it’s equally crucial to comprehend the intricate nature of the diode’s construction. The precise depths of the n and p layers, which usually vary between 20 to 100 μm, play a critical role in determining both the device’s voltage capacity and its switching speed.
Recent innovations in the manufacturing of DSRDs, specifically in Silicon epitaxial (epi-Si) growth technologies, have pushed the boundaries of their performance. This advancement has enabled the creation of diodes with thicker layers while maintaining precise control over the layer’s doping concentration. When amalgamated with meticulous modeling, this technology has yielded DSRDs with unparalleled switching performances.
However, despite their impressive functionalities, challenges persist in the realm of DSRD technology. For instance, although individual DSRD dies can generate pulses with astounding rise rates, stacking multiple DSRDs to amplify the output voltage often encounters issues of saturation. Another significant challenge is the dynamic characterization of large DSRD stacks that can handle high current densities to generate voltage pulses surpassing the 1 kV mark.
The drift step recovery diode stands as a testament to the unyielding march of semiconductor technology, offering unprecedented pulse generation capabilities. As research continues and technology advances, it is anticipated that solutions to current limitations will emerge, further cementing the DSRD’s position as a cornerstone in high-frequency, high-voltage applications.
BXDP 83 and BXDP 84
The BXDP 83 diode stands out as a distinctive silicon mesa-type charge diode, characterized by its thermal oxide passivation over the active region. This specialized passivation ensures enhanced stability and reliability of the diode. Encased in either an 0C4 or 0C4p ceramic-metal housing, this structure provides robust protection to the internal components, ensuring longevity and resilience against external factors. Given its design specifications, the BXDP 83 diode finds its niche in harmonic generators and pulse shaping circuits, allowing it to efficiently handle high-frequency operations and produce consistent output waveforms.
Similarly, the BXDP 84 diode belongs to the family of silicon mesa-type charge diodes, mirroring some traits of the BXDP 83. Its active area benefits from the thermal oxide passivation, which bolsters its performance by minimizing undesirable interactions and enhancing its operational lifespan. Enclosed within the durable 0C4 or 0C4p ceramic-metal housing, the BXDP 84 diode is shielded against potential environmental threats, ensuring a consistent performance. Tailored specifically for harmonic generation, this diode excels in converting input signals into multiple harmonic frequencies, making it a valuable asset in radio-frequency and signal processing applications.
A mesa-type diode is a semiconductor device that undergoes a specific fabrication process, wherein the active region of the diode is elevated, resembling a plateau or “mesa”. This unique design comes from etching away parts of the semiconductor layers, leaving a raised structure. The mesa configuration offers several advantages: it provides better control over the junction area, reduces capacitance, and can enhance the diode’s high-frequency response. Originating from early semiconductor technology, mesa-type diodes were one of the solutions to challenges faced in creating reliable and efficient pn junctions. While newer manufacturing techniques have emerged over the years, mesa-type diodes still find applications in specialized areas due to their distinct characteristics and performance attributes.
Advantages and disadvantages of Step Recovery Diode
Step Recovery Diodes (SRDs) are specialized semiconductor devices that come with their own set of advantages and drawbacks, tailored to specific applications. One of the principal benefits of SRDs is their ability to produce forward current more swiftly than traditional diodes. This rapid current generation, combined with their fast switching time, ensures a prompt response, making them particularly valuable in applications requiring swift transient responses. This is further complemented by their aptitude to produce exceedingly sharp pulses, pivotal for precise pulse generation tasks. In addition to these, SRDs are celebrated for their high efficiency, streamlined design, and cost-effectiveness. The reduced power loss and minimal reverse recovery time contribute to their high-performance metrics, making them an attractive choice in various electronic configurations.
However, like all components, SRDs are not without their limitations. The most notable challenge associated with them is their performance degradation at elevated frequencies. As the frequency ascends, the switching speed of the diode wanes, limiting its application in high-frequency domains. Another potential constraint arises when there is a significant discrepancy between input and output levels; under such conditions, the efficiency of the diode can be compromised. Moreover, due to their inherent design and the intensity of operations they’re subjected to, SRDs might necessitate the integration of a heat sink to dispel excess thermal energy. It’s also worth noting that SRDs are predominantly designed for step-down operations, restricting their versatility in certain circuit topologies.
To expand on the subject, it’s essential to understand the environments where SRDs shine. Their quick transient response makes them apt for pulse generation circuits, RF applications, and waveform shaping. However, for applications necessitating operations at very high frequencies or those requiring step-up functionalities, alternative diode types might be more suitable. As technology evolves and the quest for perfect components continues, it’s crucial for designers and engineers to weigh the pros and cons of SRDs, ensuring their optimal integration into electronic systems.
Step recovery diode applications
Step Recovery Diodes (SRDs) have found their niche in a wide spectrum of applications, predominantly due to their unique characteristics and behavior under varying conditions. Their primary ability to act as frequency multipliers, potentially multiplying the input signal frequency by a factor of up to 20, stems from their rapid switching time and charge storage capabilities during forward biasing. When reversed biased, this stored charge is leveraged to produce harmonics, leading to the generation of sharp, distinct pulses. This ability to create such pulses facilitates the use of SRDs in harmonic generation, producing a range of pulses tailored for specific applications.
One of the key attributes that sets SRDs apart is their high cut-off frequency range, spanning between 200 GHz to 300 GHz. This extensive range offers the flexibility to implement them in circuits operating close to a whopping 10 GHz, further establishing their dominance in high-frequency applications. In addition to frequency multiplication, the diode’s proficiency in generating sharp pulses lends it to be a perfect candidate for harmonic generators, enabling the production of varied pulses that cater to specific requirements.
Furthermore, the SRD’s adaptability doesn’t stop there. In the realm of oscillators, SRDs are integrated into Voltage-Controlled Oscillators (VCOs), which are fundamental building blocks in many RF systems. Their inclusion in frequency synthesizers also underscores their role in precision frequency generation and control. The “Comb Generator” application is particularly intriguing, given that the output waveforms of such devices mimic the appearance of comb teeth, giving them their distinctive name. On the communication front, their deployment in sampling phase detectors aids in discerning the phase in communication systems, proving invaluable in signal modulation stages.
Beyond the applications outlined, it’s essential to recognize the potential future applications of SRDs. With the continual evolution of communication systems, especially in the 5G and potential 6G arenas, the demand for devices that can handle high frequencies and offer sharp, clear signals is bound to increase. SRDs, with their intrinsic capabilities, are well poised to be at the forefront of these technological advancements. As research progresses, it wouldn’t be surprising to see SRDs integrated into more sophisticated, next-generation systems, potentially shaping the future of communication and signal processing.
Step recovery diode researches
Recent advancements in the realm of step recovery diodes (SRDs) have focused on enhancing their performance in high-voltage and high-frequency applications. A notable development is the 4H-SiC drift step recovery diode (DSRD) with a super junction structure, which is advantageous for high-voltage applications due to its non-requirement for deep etching and higher saturated drift velocity in the n-type drift layer. This structure has been identified as more beneficial than the traditional structures for high-voltage DSRD applications.
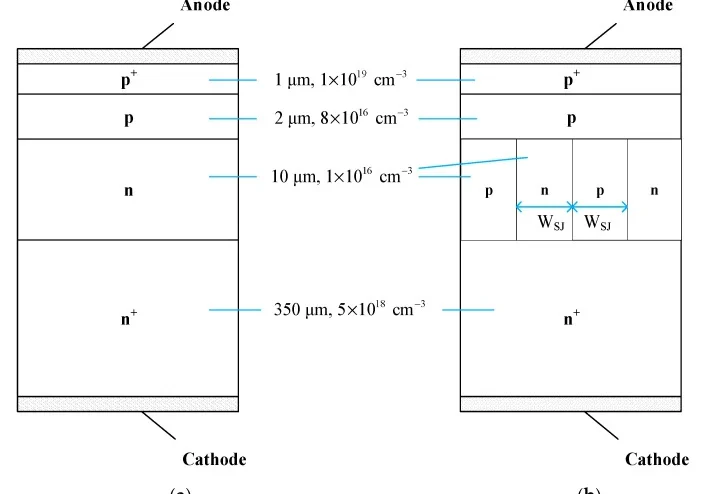
Furthermore, research has been conducted to optimize SRD pulse circuits for use in impact radars. By analyzing Gaussian waveforms and simulating pulse generation with various SRD structures, researchers aim to simplify circuits and improve the detection performance of fuzes, choosing waveforms that are particularly suitable for shock pulse emission.
In the field of ultra-wideband applications, a significant contribution has been the design of ultra-short picosecond pulse generators using SRDs. These developments include the analysis of equivalent circuit models of SRDs, leading to improved nonlinear simulation programs that are integrated with circuit emphasis (SPICE) models for accurate simulation in Advanced Design System (ADS) software. Another paper highlights the creation of a high-performance, low-ringing ultra-wideband monocycle picosecond pulse generator using an SRD, a field-effect transistor, and a Schottky diode, showcasing practical implementation of the technology.
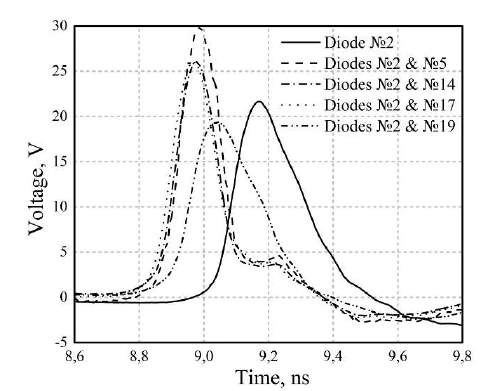
Further studies have improved the performance of ultrashort pulses by serially connecting step recovery diodes, highlighting the role of silicon diodes such as DSRDs and SOS diodes in achieving high current densities and power off capabilities. DSRDs, specifically, have been harnessed in pulsed-power generators to produce nanosecond-scale pulses with impressive rise rates.
The operation and characteristics of high-power SRDs have also been detailed, describing their dual-phase reverse recovery process that is essential for their step recovery behavior. This is crucial when SRDs are used cyclically to maintain the minority charge for efficient operation.
Finally, a new SRD model for computer-aided design (CAD) has been developed, taking into account the voltage ramp in the SRD and using direct current (DC) measurements to extract model parameters. This model facilitates the use of commercial circuit simulators for the design of SRD circuits, with practical applications analyzed for comb generators and frequency multipliers.
These research findings represent a leap forward in the application of step recovery diodes, addressing the demands of modern electronic systems for faster and more robust components. The integration of SRDs in complex circuits for enhanced performance is a testament to the ongoing innovation in semiconductor technology.