SQUID stands for superconducting quantum interference device. And this is probably most important superconducting device in the world today. The are three key quantum mechanical effects crucial to the operation of the SQUID:
- Superconductivity: This phenomenon, where materials exhibit zero electrical resistance and the expulsion of magnetic fields when cooled below a certain critical temperature, is a major focus of ongoing research. Superconductors are foundational in the field of quantum mechanics and continue to attract significant scientific attention due to their unique properties and potential applications.
- Josephson Effect: Discovered by Brian D. Josephson, this quantum mechanical phenomenon occurs in superconductors and is characterized by the flow of an electric current between two superconductors separated by a very thin insulating barrier. This effect is integral to the operation of SQUIDs, as it allows for the precise detection of extremely small changes in magnetic fields.
- Magnetic Flux Quantization: Analogous to the quantization of angular momentum in electrons orbiting atoms, this principle is critical to the functioning of a SQUID. It involves the quantization of magnetic flux in superconducting loops. The key aspect here is phase coherence, where the phase of the superconducting wave function must be consistent around the loop, allowing the SQUID to detect even minuscule changes in magnetic flux.
SQUID Superconducting quantum interference device is a very sensitive, weak magnetic field meter. The device is built using superconducting loops with Josephson junctions. Josephson junction was designed upon the theory of B.D. Josephson (b. 1940) was the author of an article published in 1962. Josephson discussed how current can be flowed between two superconductors that are separated by a thin layer of insulator. The effect is now referred to by the Josephson effect. The SQUID is made up of an ultraconducting loop as well as the two Josephson junctions. The currents flowing through these connectors increase and cause interference. When the loop is within even a small magnetic field outcome of the interference may be different because of the shift in phase between the currents induced due to the magnetic field.
The term SQUID refers to an abbreviation of its full title: Superconducting Quantum Interference Device. Superconducting signifies this SQUID is made from superconducting materials, that is, material with zero electrical resistance and is able to conduct current with no loss. What is known as Quantum Interference is much more obscure and is connected to the physical foundation for the SQUID. It is essentially a device or instrument. In this category is an electronic device that can measure the field of magnetic energy with precision which is unattainable with other sensors.
Construction of SQUID
It is made from superconducting wire that is connected to form a ring. In two locations, the circuit is cut and divided by an insulator layer insulation. This creates two superconductor-insulator-superconductor junctions, called Josephson junctions. The entire system is completed by two leads, which link both the SQUID to the electronic circuit.
But, creating a high quality circuit isn’t that easy. The typical loop of an SQUID is less than 0.1 millimeters in surface area, and is composed from pure Niobium. The junctions are constructed of niobium oxide. They are around 1 nanometer in thickness and comprise only the atoms of a few layers. This means that the whole process is typically made by the process of lithography just like semiconductor processors are manufactured. A completed SQUID is available for purchase as any other electronic component, though the costs are rather high. For Oxford Instruments, for example the chip made using superconductors at low temperatures costs around $3000; to buy one that is made of high-temperature superconductors, you will pay around $7000. The prices don’t include the electronics required that cost about a tenth of a second more.
SQUID Operating principle
The mechanism of SQUID is built on the concept of interference, similar to the interfering of light in the famous experiment of Young’s two slits. In SQUID however the interference does not occur between two light beams however, but rather between the two wave acts in the two superconducting portions of the rings. In both instances, the effect is the same and the degree to which the interference is destructive or constructive varies on the mutual frequency of the two waves. In SQUID it is the frequency of the waves that are correlated to magnetic fields flowing across the loop.
The total flow of current I through the SQUID with a constant voltage, varies according to the field of magnetic. In the event that the magnetic Ph that flows across the loop (that is the product of the field induction and the size that the loop) is an integer multiple of the quantum flux
then the current is the maximum. If
Then, minima are seen. This causes an oscillation in the current in relation to of the field external to it. The curvature’s envelope is a result of diffracted light that is related to the dimensions of Josephson junctions, which is similar to the way light scatters across the slice.
Magnetic field sensors
Based on the result that was previously described magneto-field sensors are now being designed with sensitivities as large as a few Femtotesla (10-15). In real-world applications the magnetic field measured is not directly transmitted via the loop of SQUID instead, a superconductor circuit is utilized. It is constructed to determine the magnetic field or it could be wound in order to serve as a gradiometer. That is, to determine the change in the spatial field. The magnetic field changes and creates a constant current within the superconducting circuit which then creates an electric field within the coil. It is the field that is detected through the SQUID. The SQUID’s features allow for measurements of weak, changing signals in a large but steady field.
Difficulties in SQUID
The main problem with using SQUIDs (Superconducting Quantum Interference Devices) is that they need to be made from superconductors. Unfortunately, despite many years of research, we haven’t found a material that can superconduct at room temperature. The best SQUIDs work only at very low temperatures, below 10 Kelvin (-263°C). This requires cooling them with liquid helium, which is expensive.
In recent years, SQUIDs made from high-temperature superconductors (HTSCs) have been developed. Despite their name, they also need cooling, but only to temperatures below -150°C. For this, liquid nitrogen, which is cheaper than helium, is sufficient. These liquid nitrogen-cooled devices are portable and useful for certain tasks like measuring geophysical properties.
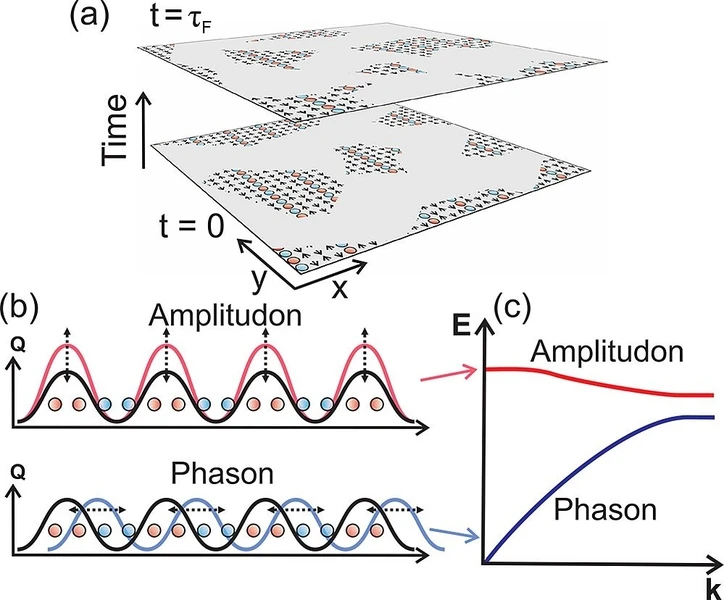
However, HTSC SQUIDs are less sensitive than those cooled with helium. Another challenge with SQUIDs is interference from nearby electrical sources, like wires, motors, transformers, and the Earth’s own magnetic field. This interference can be stronger than the signals from the objects being studied. To reduce this, shields made from special materials are used. Mu-metal, which is highly responsive to magnetic fields, is commonly used to block slower-changing fields. For rapidly changing fields, shields made from strong conductors work well, as they generate currents that block the magnetic field. In extremely sensitive measurements, both the sensor and the subject are placed inside a room with thick shielded walls to block external magnetic signals.
Superconducting quantum interference device instruments and applications
Magnetoencephalography
Measuring very low magnetic fields has useful applications not only in physics but also in geophysics and medicine. A key use is in magnetoencephalography (MEG), which tracks brain activity by measuring the magnetic fields created by nerve impulses. The magnetic field from a group of neurons can reach up to 500 femtotesla, even through the skull. MEG uses hundreds of sensors cooled to the temperature of liquid helium and protected by a heat shield. The patient and the device are placed inside a room surrounded by heavy magnetic shields. The sensors send signals outside to be amplified, recorded, and analyzed by special software.
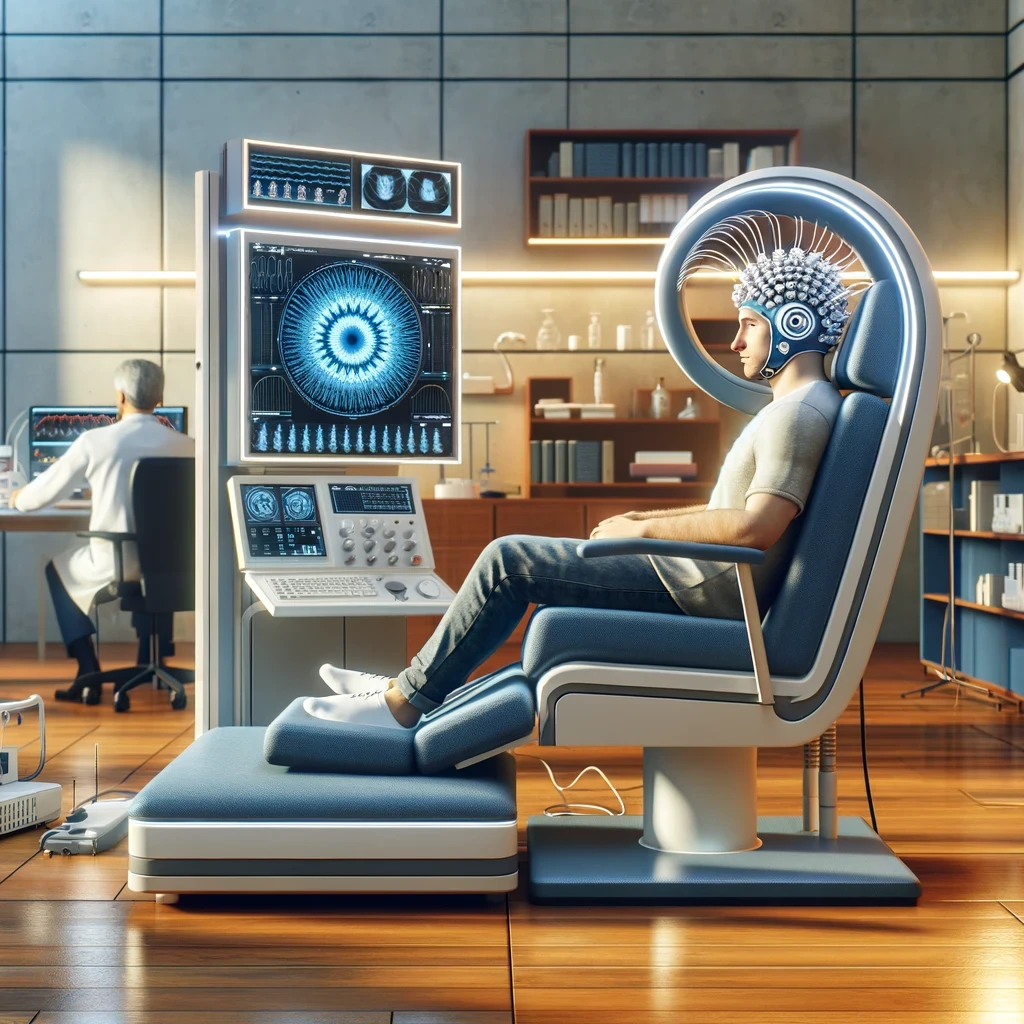
MEG data is different from what we get from magnetic resonance imaging (MRI) or tomography. MRI and tomography show us the structure of the body, but MEG shows how organs function. MEG can track brain responses to things like sight or sound within 1 millisecond, allowing us to see brain activity in real-time. It’s also precise in locating which part of the brain is active. This helps us understand which areas respond to visual or auditory signals, and locate regions related to memory and speech
Geophysical research
In certain rocks, information is recorded on the direction of Earth’s magnetic field was at the time the rock was solidified. The reason for it is the following. Iron at temperatures higher than 770oC is paramagnetic, and therefore cannot create a magnetic field on its own. If the iron-rich rock is cooled below 770oC it becomes ferromagnetic. Its spontaneous magnetic field follows its direction from the field externally. The whole rock is an inert magnet. Today, geologists can obtain rock samples making sure to record their location relative to regions of the globe and in a laboratory, they can determine by using a magnetometer which direction is the magnetization for the sample. It is apparent that earth’s magnetic field hasn’t always been the way it is now. Over the course of 70 million years the magnetic poles changed about 170 times. Today, it remains unclear what leads to such a switch.
New materials, magnetometers
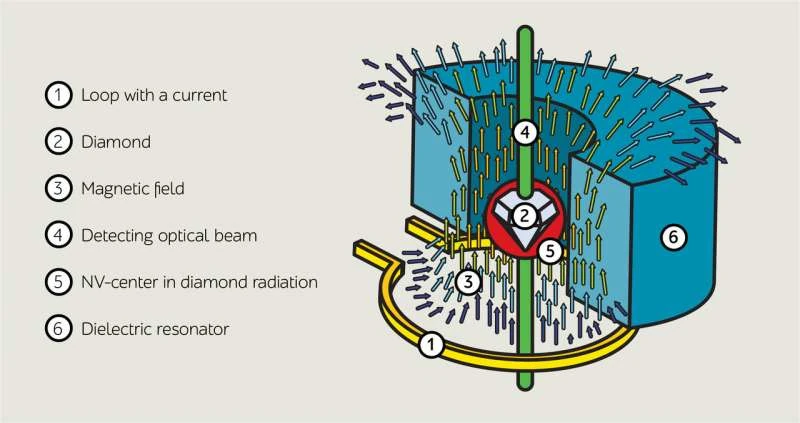
Scientists are discovering and creating a wide range of exciting new materials. These include both newly made chemical compounds and tiny manufactured structures. In studying these materials, magnetometers are often essential. The importance of magnetic properties is evident in everyday items. For example, the way CD-ROMs work and the construction of Walkman headphones rely on recent materials with specific magnetic properties. Magnetometers are tools used to measure these magnetic properties.
SQUID on spy duty
Finding enemies (or allies) submarines is a problem for intelligence services for many years. One method for detecting vessels is to measure the strength of the magnetic field on Earth by a satellite with low flight. Submarines, just like all ferrous objects, alter the magnetic field that is in the vicinity. The alteration of the earth’s magnetic field is significant enough that it is observable at a great height with an SQUID-based magnetometer. In the ocean ships are the only big iron structures. So, if a perturbation of earth’s fields is occurring while there is nothing visible at the top of it, this means that something is underground. The research on these techniques is classified, and it is difficult to determine if it is true or not.
Importance of superconducting quantum interference devices
Superconducting Quantum Interference Devices (SQUIDs) play an enormously crucial role due to their unique properties and capabilities. Here are a few reasons why SQUIDs should be given greater consideration:
1. High Sensitivity: SQUIDs are highly sensitive magnetometers capable of measuring even very weak magnetic fields – down to 1015 Tesla! They make SQUIDs indispensable tools in many scientific and technological applications.
2. Non-destructive Measurement: SQUIDs provide non-destructive magnetic field measurements without direct contact to objects being measured, making them suitable for applications such as biomagnetism where measuring brain activity or detecting small magnetic signals from within humans is a necessity.
3. Broad Frequency Range: SQUIDs can operate across an extensive frequency spectrum, enabling them to measure both static and dynamic magnetic fields. DC SQUIDs excel at low frequency applications like geophysics and material characterization while rf SQUIDs offer precise magnetic field measurement in rapidly shifting fields such as nuclear magnetic resonance (NMR) imaging or magnetic resonance imaging (MRI).
4. Quantum Sensing: SQUIDs are devices based on superconductivity and quantum interference principles, making them an excellent example of quantum sensing devices. Utilizing the quantum properties of superconducting materials and Josephson junctions as conduits into quantum space, SQUIDs offer unique windows into this realm that make them valuable tools for researching fundamental physics phenomena as well as quantum computing research.
5. Diverse Applications: SQUIDs have many different uses across many disciplines. Physics researchers utilize SQUIDs for researching superconductivity, magnetic properties of materials and quantum phenomena; SQUIDs can be utilized in magnetoencephalography (MEG), which maps brain activity to diagnose neurological disorders; they’re even employed geophysics research for mapping underground mineral deposits and studying Earth’s magnetic field variations as well as nondestructive testing, quality control and industrial applications.
6. Scientific Discoveries and Technological Advancements: SQUIDs have played a significant role in many scientific breakthroughs and technological developments. Their contributions include breakthroughs in understanding superconductivity, magnetic phenomena and quantum effects as well as aiding medical diagnostic techniques and magnetic field sensing imaging technologies.
SQUIDs have immense importance because of their extraordinary sensitivity, quantum nature and wide applicability; making them invaluable tools across scientific, medical and industrial domains.
Research studies including SQUID devices
Quantum Hall-based Superconducting Interference Devices
The research on Quantum Hall-based Superconducting Interference Devices (SQUIDs) represents a significant advancement in the field of condensed matter physics and quantum computing. This study, conducted by a team including Andrew Seredinski, Anne W. Draelos, Ethan G. Arnault, Ming-Tso Wei, Hengming Li, Tate Fleming, Kenji Watanabe, Takashi Taniguchi, François Amet, and Gleb Finkelstein, was published in Science Advances in September 2019.
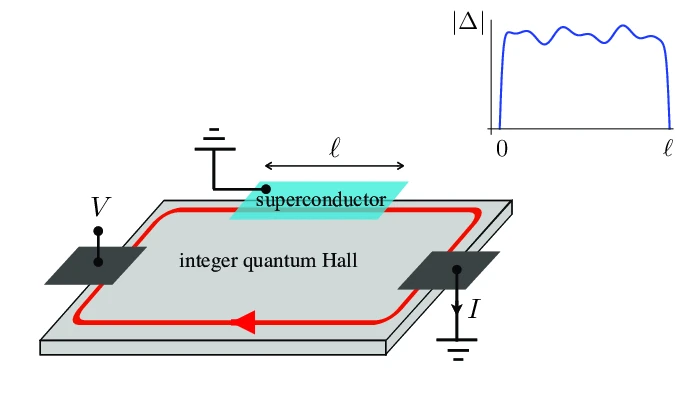
The study focuses on a graphene-based Josephson junction, which is a critical component in superconducting quantum interference devices. What sets this research apart is the use of dedicated side gates, which are carved from the same sheet of graphene as the junction itself. These side gates are highly efficient and allow for modulation of carrier density along either edge of the junction over a wide range.
A significant aspect of the research is the exploration of supercurrents in this system, especially in the context of magnetic fields ranging from 1 to 2 Tesla. This magnetic field range enables the population of the next Landau level, leading to Hall plateaus with conductance that differs from the bulk filling factor. When counter-propagating quantum Hall edge states are introduced along either edge, a supercurrent localized along that edge of the junction is observed. This phenomenon is studied as a function of magnetic field and carrier density.
The research team’s findings are important for the exploration of correlated and fractional quantum Hall-based superconducting devices. These devices are proposed to host non-Abelian Majorana and parafermion zero modes, which are of great interest in quantum computing and advanced magnetic field sensing technologies.
Moreover, the study details the interference patterns observed in these devices. The differential resistance map versus various gate voltages reveals suppressed resistance due to the supercurrent, indicating the presence of a supercurrent on top of quantized plateaus. The research also delves into the sensitivity of the supercurrent to changes in the magnetic field, showcasing SQUID-like interference patterns under certain conditions.
This research contributes significantly to our understanding of superconducting quantum interference in quantum Hall regimes and has potential applications in developing advanced quantum devices and technologies.
Superconducting Quantum Circuits for Quantum Information Technology
Recent advancements in superconducting quantum circuits for quantum information technology have led to significant progress in quantum computing. Superconducting quantum circuits have become a prime contender for implementing quantum processors, aiming to realize universal quantum computing. These advancements are evident in the increased scale and quality of quantum processors, as measured by the number of quantum bits (qubits) connected on a chip and by metrics like quantum volume.
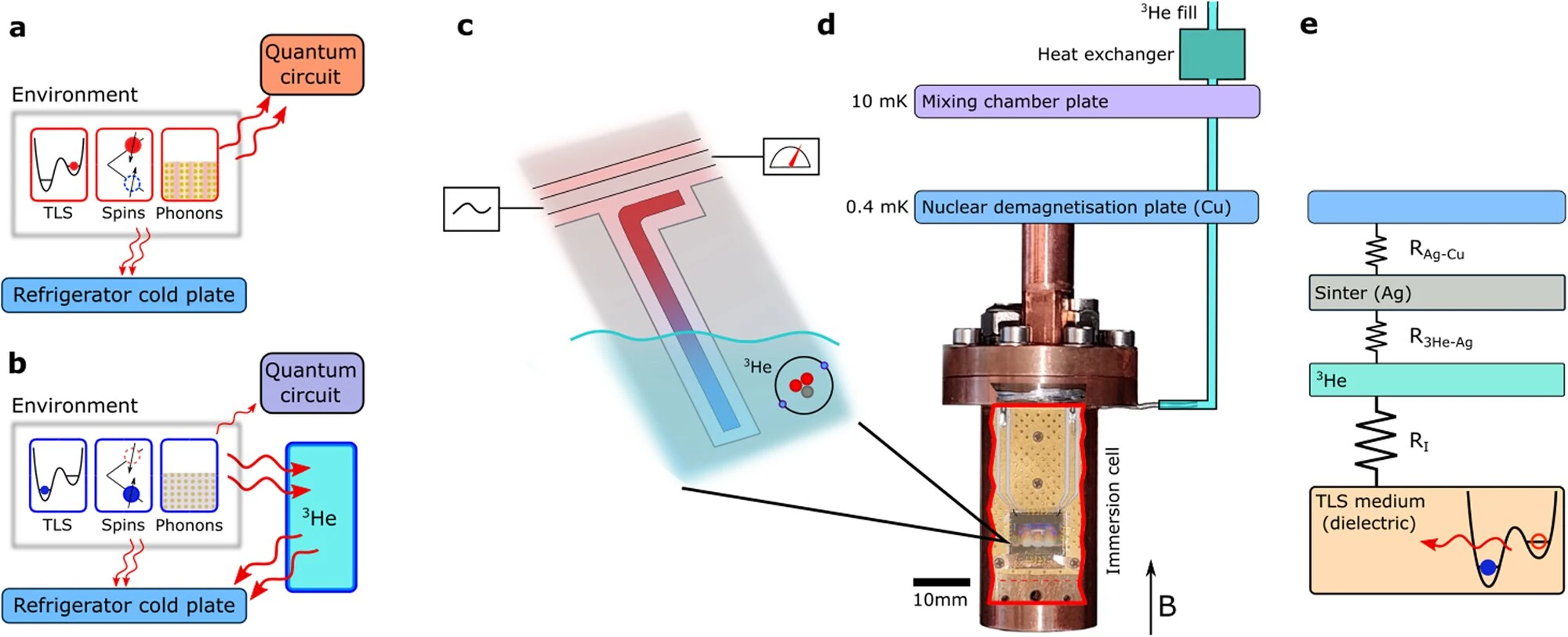
One of the key aspects of these circuits is their design and manufacturing process. Superconducting quantum computing devices are typically designed within the radio-frequency spectrum, cooled in dilution refrigerators below 15 millikelvins, and addressed with conventional electronic instruments like frequency synthesizers and spectrum analyzers. The manufacturing process involves advanced techniques such as lithography, metal deposition, etching, and controlled oxidation. Continuous improvements in these processes have significantly enhanced the lifetime and performance of superconducting qubits since the early 2000s.
In terms of technology, superconducting quantum circuits have been employed to develop highly sensitive thermometers. For example, defects within a nanodiamond, such as nitrogen-vacancy (NV) centers, have been used alongside flux qubits to measure magnetization changes due to temperature variations. This approach offers a high-sensitivity figure of merit at ultra-low temperatures, providing a more compact and efficient method for temperature measurement in quantum systems.
Furthermore, the research on bosonic qubits in superconducting quantum computers is progressing steadily. This is highlighted by significant announcements, such as Google’s 2019 demonstration of “quantum supremacy” and advancements in the field of fault-tolerant quantum computing. Despite these achievements, reaching full fault-tolerant quantum computing still requires overcoming substantial hardware overhead and performance requirements for error-correction codes.
Overall, the field of superconducting quantum circuits for quantum information technology is rapidly evolving, with ongoing research and development poised to make further significant contributions to the realm of quantum computing.
References:
http://www.foton.if.uj.edu.pl/documents/12579485/e462c02f-4346-4089-befa-8a9852845e07
https://openstax.org/books/fizyka-dla-szk%C3%B3%C5%82-wy%C5%BCszych-tom-2/pages/9-6-nadprzewodniki